Flash and JavaScript are required for this feature.
Download the video from iTunes U or the Internet Archive.
Description: In this lecture, Prof. Liu first finishes discussing class string dynamics in the light-cone gauge, and then proceeds to quantize the theory in the light-cone gauge.
Instructor: Hong Liu
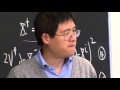
Lecture 11: String Theory i...
The following content is provided under a Creative Commons license. Your support will help MIT OpenCourseWare continue to offer high-quality educational resources for free. To make a donation or to view additional materials from hundreds of MIT courses, visit MIT OpenCourseWare at ocw.mit.edu.
PROFESSOR: No, no minus sign. I think maybe-- yeah, it should be a plus sign. Yeah. Should be a plus sign. Yeah. Yeah, you should correct that minus sign to a plus sign. Yeah, both should be plus sign. Yeah-- I wrote on my notes was correct, but then on this board, somehow-- [INAUDIBLE] maybe I should have a minus sign and put it there. But didn't realize.
Yeah. So let me first remind you what we did in last lecture. So first, we have some gauge symmetries on the [? worksheet. ?] And then we can use that, say, [INAUDIBLE] traditional symmetry. You can re-parametrize the [? worksheet ?] coordinates, and also to a [? while ?] scaling for the metric itself. Use those freedom, we can set the metric to, just to the flat Minkowski metric. [? On worksheet. ?]
Now after you do that, then the [? worksheet ?] action then becomes just like a free scalar field. Then of course, the equation of motion is just given by the standard [? wave ?] equation. I then can solve it immediately. And essentially, you just have some left-moving wave, some right-moving wave, and plus, some zero modes.
And then for a closed string, these are independent functions. So then for the closed string, closed, so you can have independent left-moving modes, and then also independent right-moving modes. And but for the open stream, because you have boundary conditions, and then you only have one set of modes, because you are-- yeah, because boundary conditions give you a constraint. So it's just like you have a standing wave. And then X l should be equal to L r. Yeah, X l should be equal to X r.
But this is not-- but [? quantized ?] string theory, even in this gauge, is not just quantized scalar field theory, because we still need to solve the equation motion come from doing the variation of gamma a b themselves. And the way you do the variation of gamma a b themselves, they see [INAUDIBLE] to set the stress tensor of this scalar field theory zero. Yeah, to set the zero, the stress tensor.
And so, already, just-- yeah, so they're two independent equations. Y is the diagonal component. It should be zero. And then the other is the off-diagonal component, should be zero. So you have two sets of equations. And those equations are in general hard to solve. So they are non-linear quadratic equations. Which I did not write them here. So they're non-linear constrained equations, which are, in general, hard to solve.
Then the important trick we discussed is that you can go to the light-cone gauge. We said even after this fixing, there's still some remaining gauge freedom. That you can actually make one more choice to set the X plus, set it to be the same as tau. And the X plus, we always use this definition. So you can see that the all directions, X zero, X one, two, et cetera. And then we combine X zero and X one together to form X plus X minus. And then the rest we call them X i. So these are also sometimes called transverse directions. So also called transverse directions.
And so now, then we can use this remaining gauge freedom to go to so-called light-cone gauge. Then the X plus becomes V plus times tau, some constant times tau. And then this light-cone gauge, then those equations can be written in a very simple way. In particular, the dependence on X minus becomes linear. Then you can actually use these two equations-- you can solve X minus exactly. And so this tells you that actually, the independent [? degree of ?] freedom just X i's. OK? The independent [? degree of ?] freedom just X i's. Any questions about this? So let me just put the equation number, because I'm going to use this equation number later. Which inherited from the last lecture.
AUDIENCE: So the-- [? confidence ?] on the [? energy ?] [INAUDIBLE] because they're [? close together ?] [INAUDIBLE]
PROFESSOR: No. No. This come from the equation motion for gamma a b. So [? remember, ?] previously, the gamma a b is also a dynamic variable. Even when you fix the gauge, you still impose the equation motion. And so that's essentially this. Yes?
AUDIENCE: Aren't the remaining degrees of freedom for X minus just because of the boundary condition, or something like that? Is there a freedom in specifying that?
PROFESSOR: What-- There's some constant, a [? possibility ?] constant and we can-- of all constants. Yeah, because this determines all the derivative dependents. And they might be, overall, constant. And that you can always-- yeah, it's not important. Yeah, yeah. Any other questions? Yes?
AUDIENCE: So going back to what you said earlier. So for the boundary conditions for the open string, the derivative's also positive? So there's not a minus sign?
PROFESSOR: Yeah. Let me also add here-- so for the open string, for the moment, we can see the so-called Neumann boundary condition. So this is equal to zero. So that, we'll keep that. Yeah, so one correction. In the last lecture, I wrote a minus sign there. That should be corrected by plus sign. The both equations should be corrected by plus sign. Yeah. Good?
So with this set up, then we are ready for quantization. Because now, we only need to quantize the X i's, because X i's are independent-- only X i's are independent variables. And so we only need to quantize X i's. And X i's, they are just a free field. And that's very simple. OK?
But before do that, let me just emphasize one point. Emphasize one point. So the light-cone gauge, by making this choice, you make those equations very simple, but you also sacrifice something. Because of the light-cone gauge, because the [? shaky ?] choice itself, it breaks Lorentz symmetry.
OK? Because [INAUDIBLE] [? it lacks ?] two special directions. So X zero X one, and you form X plus from them, and you impose some conditions on X plus. So this breaks Lorentz symmetry.
OK? So when we say "break," this does not really break the Lorentz symmetry. Just say this gauge choice itself does not respect Lorentz symmetry. OK? So the theory is still Lorentz symmetric. The theory should still have Lorentz symmetry. It's just Lorenz symmetry not manifest. Say, yeah, say just not manifest. Yeah, maybe I should-- using proper English, I should say, in the light-cone gauge, Lorentz symmetry is not manifest. I think this is a better way to say it. OK? It's not manifest. So this equation breaks Lorentz symmetry, and then the Lorentz symmetry is not manifest.
So but there's a third group. Lorentz symmetry is still manifest. So that's the group which rotates the X i. So this remaining s o d minus two, which rotating X i's. This is manifest. OK. The remaining rotating X i is manifest. OK? So keep this in mind. OK? This is very important point.
OK. So now we can proceed to quantize it. So before doing the quantization, let me develop a little bit further to make the task of the quantization easy. So I ask, what we do in free field theory is [? continue ?] to expand to those arbitrary functions in terms of Fourier modes. OK? And they are periodic functions of 2 pi, so we can easily expand them in Fourier modes. For example, X r X l mu sigma plus tau can be written as i over prime 2. OK?
So this alpha prime, so this [? pre-factor ?] is purely convention. OK? Just for the later convenience. And this one over n is also purely convention. And you can choose what you want.
So the key is that you are expanding this periodic function. Inferior modes. Because you have to be periodic in sigma, so the coefficient here must be integer n. And we exclude unequal to zero mode, because the unequal to zero, this is just a constant. And that is already included here. And that is already included here. So we say exclude unequal to zero.
And also this is a real function. Then this means that the alpha n mu should be equal to alpha minus n. So with this convention over n here, and with this i here, would be alpha minus n mu [? ba. ?] OK?
And similarly, you can write down the expansion for X r. Similarly, you can write down the expansion for X r. The same [? pre-factor. ?] And this n sum for minus infinity to plus infinity but not equal to-- unequal to zero, so we call the modes f n tilde divided by n. [INAUDIBLE] tau plus sigma, tau minus sigma. OK? And here, again, alpha tilde mu should be equal to [? ba ?] alpha tilde mu minus n, in order this to be real.
OK. For the open string, you only have one set of modes. For open strings. So this is for closed string. For open string, you can-- you just have alpha. Yeah, just alpha and mu, you go to alpha and mu tilde. So you only have one set of modes, OK, because are they equal.
OK. So you can plug this, those expressions, into here. Then you have most general form of X mu in terms of these Fourier modes. OK? So to save time, let me not do that step. So you should try to do-- yeah, just so a trivial step of yourself, write those things into a single equation. And then that's the most general form of X mu in terms of these specific modes.
So now let me make some remarks. You might wonder, what's the implication of those zero modes here? OK? So those just describe the center of mass motion of the string. Center of mass motion of the string. It's given by-- say, you just average the motion of-- you average the motion of, say, of the string. So that gives you the center of mass motion of the string.
And all those are periodic functions. So all of those are periodic functions. So all X a or X r periodic functions. So when they integrate, they give you zero. So the only thing remaining are those zero modes. And so you just get the X mu plus V mu tau. OK?
So if you think of the center of mass of the string, that's a particle. It is a point, it's a point particle. So this essentially gives you the trajectory of the particle in terms of the proper time. So you can think of this tau as the proper time for the center of mass of the string. And then this just-- yeah. V mu is the velocity for the center of mass in terms of the proper time along the string. OK?
And so all these different alpha n, alpha n tilde-- so those just parametrize the different oscillation modes. So they just parametrize the different oscillations of the string. Yeah, of a string. Oscillation of a string. So any particular alpha n is non-zero, means that particular harmonic is present in the string motion. Et cetera. OK?
And for the closed string, as I said, before we have both left- and right-moving. Because you have left- plus right-moving waves. But for the open string, you essentially only have a standing wave, because of the boundary condition. OK? So you only have one set of oscillation modes, rather than two sets. So any questions on this? Good?
So in the light-cone gauge, we can do further. Between the light-cone gauge, we can further solve this X minus. OK? So let's try to do it classically. In the light-cone gauge-- OK. So X minus can be solved by inserting the mode expansion into this equation 14 on the 15. OK? Then you're just equating different Fourier modes, the coefficients of different Fourier modes. OK? And then you can solve V minus, alpha n minus, alpha n minus, alpha n tilde minus, et cetera. OK?
So now let's look at the zero modes. OK? So let's look at the equation satisfied by V minus. So let's look at the zero modes. So let's look at-- first look at equation 14. So if you say zero mode, means we look at the unequal to zero modes. And equal to zero modes here, partial tau X minus, you just have a V minus. So left-hand side-- so from 14-- so let's first do for the open string. So left-hand side you have 2 V [? 2 ?] minus.
So the right-hand side. So if you look-- again, you look at the mode, which is unequal to zero. So there's one obvious term come from just taking derivative of this term. And so we have a V i squared term. OK? And then you will have a contribution from oscillators. Which I will not go into details here. So you can easily calculate it yourself. So you find for the open string. That's the answer. And so there's only one set of modes. And similarly, you can do it for the co-string. Again, on the left-hand side, just 2 V plus, 2 V minus, and the right-hand side you have V i squared. But now you have two sets of modes. And also there's some coefficient difference.
So other than this [? pre-factor ?] 2 on the one, here, you can actually wrote down those expression just by closing your eyes. Just write them down. Because you have a zero mode, these two have to add up to zero. So that's the only possibility. So other than this factor of 2 in the one you need to check yourself, you can essentially write down this expression immediately, just from the structure of that equation. OK?
AUDIENCE: What's the definition of V i?
PROFESSOR: V i is there. V mu in the i direction. And V minus, V plus, it's all that.
So those equations are of great importance. And let me box them. Which is also the reason I write them down. So but now, I did not leave space to give them numbers. Yeah, anyway, these are the two equations [? of ?] add the numbers [? nature. ?]
From the 15, from the equation 15-- so this is a consequence from the 14, the zero modes from the 14. From the 15, you get nothing. You get zero equal to zero for open string. So this is obvious, because on the left-hand side, zero, because for the open string-- for the-- yeah. Yeah, because for the-- because left-hand is zero, because the zero mode does not have an [INAUDIBLE] independent sigma. So this is zero. And then you [? credited ?] a little bit checking yourself, that the right-hand side is also zero, essentially because of this structure X 0 equal to [? X y. ?] Yeah, X 0 equal to X r.
And but for closed string, you actually find non-trivial equations. You find it-- the left-hand again, is zero. And the right-hand side-- the right-hand side, if you want to find the zero mode, essentially you just integrate over the whole thing. So let me just write it explicitly. So you can write it also in terms of modes.
OK. So this equation is very interesting. So this tells you that the overall amplitude of the left-moving string, yeah, left-moving modes, when you add them together, should be the same as the overall moving, overall amplitude. Say-- yeah, in this combination of the right-moving string. OK? Yeah, they have to be balanced. So if we look at the source of this equation, and the reason the left-hand side is equal to zero is essentially due to the periodic boundary conditions. Because the periodic boundary-- for closed system, because periodic boundary condition, you cannot have any term linear in sigma.
And the periodic boundary condition, in other words, it means that there is no special point on the string. OK? Any point is the same as the other. So your choice of origin is actually arbitrary. And so this equation essentially reflects that. So the fact along the string, there's no special point, and they give you a global constraint on the oscillation of the string. OK? Between the left-moving part and the right-moving part.
And then, if you look at the-- so if you look at the nth modes, then you can find out alpha n minus, alpha n tilde minus, et cetera. OK? In the piece that you have a [? problem, ?] you will have [? found ?] doing this. Any questions so far?
So now let's look at the physical meaning of those equations. OK? I boxed them. I said they are very important. And now let's look at the physical meaning of those equations. So this can be considered as consequence of no special point on the string. And now let's look at the physical [INAUDIBLE] of those modes, of those equations. So this is the fourth comment. The third remark-- fourth remark.
So let me remind you that previously, the action have the global symmetries. So this comes from the translation. Sorry, by some constant [? m a ?] mu, all are Lorentz transformations. OK?
So those global symmetries, as we know, they are [INAUDIBLE] to [? conserve ?] the current. Conserved current on the [? worksheet. ?] OK? On the [? worksheet. ?]
So for the moment, let us consider the translation. So it's actually-- so it's a couple of lines, but I will leave you to do it yourself. I think you will also do it in your [? p ?] set. To derive the conserved current for this one. So let me just write down the answer. So the conserved current for the translation-- for translation, you can derive the conserved current because this [? t ?] such symmetry. For each mu, there's a symmetry. So the current is labeled by mu. But there's also [? worksheet-- ?] index to index, this is a current on the [? worksheet. ?] OK? So this can be written as 2 pi alpha prime. So I'm just writing down the answer, but you should-- you will check yourself in the [? piece ?] that this is the right answer. OK?
So you can immediately see that this current is conserved, because the-- if you act partial a on here, you just get the equation motion when it's zero. Equation motion just partial a squared equal to partial a squared acting on x mu equal to zero. OK? So you can immediately see this is indeed conserved. This is conserved.
And then this will lead us to a conserved charge. OK? Say, if I integrate it all along the string-- so let's do the-- for example, for the closed string, if I integrate it for the string, and this partial zero component. So the zero component, so the time component of this current, and then this is conserved charge on the worksheet. OK? So do you have any guess what should be this conserved charge equals 1 [? to? ?] Yes?
AUDIENCE: [INAUDIBLE].
PROFESSOR: Yeah. Should be, must be, the space-time momentum. OK? In your-- maybe [? level ?] one, you should have learned that-- say, if you look in the classical mechanics, for particle move with the translation symmetry, then the momentum should be conserved. OK? So here, we have a string with the translation symmetry-- moving the space time is a translation symmetry-- and then the corresponding momentum must be conserved. And this conserved charge must be that momentum. OK?
So these scenes-- so if I can see the p mu, so let me write in the p [INAUDIBLE], if you can see the p mu, which is this integration of 2 pi. So this is for the closed string. Similarly, for the open string, you integrate over pi. So this must be-- so this is the space time. So this-- oh, I'm sorry. This is a space-time momentum over the string. OK? Yes?
AUDIENCE: What's the definition of d partial a in the definition of the current?
PROFESSOR: So this is a conserved current, because [? one ?] into this global translation.
AUDIENCE: But what's d sub a?
PROFESSOR: Oh. Just the derivative of a. Derivative of sigma a. So I have always used a notation here. That the partial a is partial sigma a. So I should-- this goes to one into the space time momentum over the string. OK?
So for the closed string, you integrate from zero to 2 pi. From open string, you're not going from only from zero to pi. So now if you plug in that mode expansion to here, again, all the oscillatory modes, they should not contribute. OK? And because this is a-- you can check they do not contribute. So the only thing contribute is zero modes. So you find that this V mu, p mu, is equal to v mu divided by alpha prime. So this is a closed string. So this is [? overviews ?] from here, because this gives you v mu times 2 pi, and then 2 pi can still give you alpha prime.
But for the open string, you only integrate over pi. So you get 2 alpha prime. So this is for the open. OK? Or in other words-- or in other words, in terms of the space time momentum-- so this is a more physical quantity then this v mu-- so in terms of the space time momentum, the v mu can be written as alpha prime p mu, which is for the closed.
And 2 alpha prime p mu, this is for the open. And then this itself, of course, should be in the [INAUDIBLE] that space-time momentum density along the string. OK?
So now we have found that the v mu secretly is just the space-time momentum. OK? Essentially just space-time momentum, up to some overall constant. So now we can integrate those equations. Now we can integrate those equations. Then we can write them. So for example, for the first equation-- so now, let me give you a number. Yeah, actually, on my notes here, it's equation 18. Let me just write the equation 18 here. Only this is equation 19 for the closed. So I have more equation number in my notes than here, because I did not copy all the equations.
Anyway, so that's-- for example, equation 18. Now I can rewrite it in terms of the momentum. OK? So let me put all v to a single side. So I can write as 2 p plus p minus, minus p i squared equal to. So each p [? rated ?] 2 alpha. Under that 2 alpha here, so that's 1 over 2 alpha sum over m equal to zero alpha m i, alpha minus m i. OK? OK? So now what is this? Do you recognize what's this?
AUDIENCE: p squared.
PROFESSOR: This is just p square. Or depend on your rotation, is minus p square. OK? Minus p mu, p mu. Maybe-- let me write [INAUDIBLE]. Minus p mu p mu. So what is minus p mu p mu?
AUDIENCE: Mass squared.
PROFESSOR: Yeah it's the mass squared. So this equation should be interpreted as a mass equation. So this tells you-- so it tells you the mass of the string should be written as the form-- OK?
So now we have obtained the relation, we see that this relation-- so this is for the open string-- so we have obtained the relation between the mass of the string. So if you think about the center-of-mass motion of a string-- think, it's like a particle, then this particle can have a mass-- than this mass over the string, mass squared over the string, is related, can be expressed in terms of oscillation modes in this way. OK?
And similarly, this equation 19 for the closed string, you find that m squared equal to-- again, just differ by pre-factor-- then alpha minus m i alpha m i, plus alpha tilde minus m i, alpha m i. So this is the mass equation for the closed string. So these are typically called the mass-shell conditions. OK? So these are for the closed. Any questions on this?
AUDIENCE: [INAUDIBLE].
PROFESSOR: Hm?
AUDIENCE: [INAUDIBLE] tilde?
PROFESSOR: Sorry?
AUDIENCE: Would there be another tilde?
PROFESSOR: Oh that's right. Yeah. Thanks. Good.
AUDIENCE: [INAUDIBLE] have a question that here, before, it's a expression that we'll have to enter to find the mass of the string. So this is a kind of a definition of the mass of the str--
PROFESSOR: No, no. The definition of the mass of the string is determined by how the center-of-mass motion, right? How would you tell the [? power ?]-- the particle moves is from the center-of-mass motion. And that determines its mass. This is a conserved quantity, p is a conserved quantity, and the dispersion relation determines your mass, right? Yes, so this is the mass in the [? right. ?] Yeah. Any other questions?
Good. So now, finally, we can do the quantization, with all those preparations. OK? So now we can finally do the quantization.
So similarly, I will not go into here-- similarly, yeah, let me just also mention by looking at this transformation, you can write down the conserved [? chart, ?] conserved current [? running ?] the Lorentz transformation. Then that will give you rise to the angular momentum. And also give rise to the corresponding charge associated with the boost, et cetera. OK? And we will not go into there, they actually play a very important role understand the various aspects of the string also.
OK. So now let's-- now we can quantize it. And as we said before, we only need to quantize independent [? degrees of ?] freedom, so those X i's. So only need to quantize x i. Because these are the independent degrees of freedom. And from here, from here, you can immediately write down the canonical momentum. Say, the canonical [? worksheet ?] momentum for the string-- for the x i. So the canonical [? worksheet ?] momentum.
So I should distinguish this with this guy. So that is the-- this goes one into the current, goes one into the space-time momentum. And this is just treating so this is just treating the x i as a two-dimensional field. We can write down its [? worksheet ?] canonical momentum. And we just take the time derivative of that action for the i direction, and then you find it's given by 2 pi alpha prime partial tau x i. OK? So this is the standard to the canonical momentum for the two-dimensional field series.
So this happens to agree. So this happens to be the same as that momentum. But you should keep in mind that the physical interpretations were a bit different. There, it's corresponding to the density for the space-time momentum, and here, this just goes one into the canonical momentum, into the quantization. OK?
So now you've found the canonical momentum, then we can just impose the quantization condition. You can just impose quantization condition. So now, we will-- you promote all this as operators, all the classical field is promoted as operators, and then you imposes a canonical quantization condition. So x i x j, for different x sigma prime, but evaluated same tau, should commute. You commute. Same thing if you do the pi at a different sigma prime, but tau should commute.
But x i sigma tau and the pi j sigma prime tau then should give you i delta i j, then delta function sigma minus sigma prime. OK? So this is just the-- you just impose the-- this a free field, you just impose a standard canonical quantization condition as a two-dimensional field.
And now all of these modes, all these different modes-- oh, which I just erased half of it-- and all these different modes-- so x i p i-- so p i-- v i is the same as v i-- and then alpha n i, alpha n tilde i, they are all operators. So classically, because one into integration constant for the equation of motion, and then quantum mechanically, they become integration constant for your operator equation. So they're just constant operators quantum mechanically. OK?
And then when you plug in those mode expansions into here, just as usual, in your free field theory quantization, then you just find the accommodation relation. For example, you just find x i p j equal to i delta i j. Then you also find alpha m i alpha n j equal to alpha m tilde i alpha n tilde j equal to m delta i j delta. So I'm just writing down the answer for you. OK? So this is all straightforward, other than unconventional organizations.
AUDIENCE: So that's things like delta m minus n, or something?
PROFESSOR: Yeah, that's right. Yeah.
So now if you look at this, OK, if you look at this, so this is m. So this m is related to this normalization we were choosing here. Here we are choosing n. [INAUDIBLE] here, there's appearing some m here. OK? And from this scene, you can see-- so these all others all other commutation relation, all other commutators vanish. OK. So these are the only non-vanishing ones.
So if you look at this equation. So let's see for m greater than zero, so for m greater than zero, then this is m, then this is only [? non-manageable ?] for m equal to m equal to zero. So this alpha m alpha minus m, then you go to m something. It's a positive number. So that means, tells you, that in terms of a standard location, square root m alpha m i should be interpreted as the, say, the standard [INAUDIBLE] operator. And the square root m alpha minus m i should be interpreted as a i dagger. So this is all for m greater than zero. OK? Similarly for the tildes. Similarly for the tildes. So that's what the equation means. OK? So it's clear to you?
Then we essentially-- then we have essentially finished the quantization of the string. OK? We have solved the Heisenberg equations. And so, this is now-- we saw those modes [? expression ?] plug in. This is now interpreted as to the solution to the Heisenberg equations. And then the integration constant in these equations, they satisfy those commutation relations. They satisfy those commutation relations. And also, the commutation relation between x i and the p, indeed is it what you would expect for point particle. So this goes one into the center-of-mass location and the center-of-mass momentum. And indeed, you would expect between the position and the momentum. OK? Any questions? Yes?
AUDIENCE: Are we dropping the index m on the [? eight? ?] Or is that a subscript?
PROFESSOR: Yeah. But then we always use the notation of alpha. I will not use a. Yeah, but just keep in mind that their relation is like that. And then a and a dagger will have the standard commutation relation equal to 1. OK?
So now, we can now work out the spectrum. So now we can work out the spectrum. Let me see. So we can work out the spectrum. So the lowest state is so-called oscillator vacuum. So when we quantize it, we define our vacuum. So the vacuum [? does ?] run into, of course, the states, which are [INAUDIBLE] by all [INAUDIBLE] operators. [INAUDIBLE] by all our [? relational ?] operators. OK? Greater than zero and i.
OK. So we first define-- so in order to-- so we are quantizing-- so this is like a free field theory, and so we define our vacuum, which is a [INAUDIBLE] by all [INAUDIBLE] operator. But the difference from the standard quantum fields theory, two-dimensional quantum fields theory, is that now, the vacuum here is labeled [? by ?] p. OK? This is the space-time, center-of-mass momentum of the string. So we are taking the vacuum to be, say a momentum [INAUDIBLE] state. OK? In terms of space-time. And so this zero p, which because one and two have low oscillator exactly on the string, but can still have a momentum, a space-time momentum, so this is labeled by center-of-mass momentum. OK?
And then you can also build up other state. Then you can-- so this is the lowest state, on the-- so this is, in some sense, the vacuum state on the string. And then you can also build up the excited state. And so you just elect arbitrary number of alpha and alpha tildes. On this vacuum state. OK? So yeah, let me also label the equations. So let me call this equation 20. OK?
So for the open string-- yeah, so this is for the closed string. For the open string, you only have one set. You just have one set of, say, alpha n i. And you still have two sets. OK? It's also convenient to define oscillator number. OK?
So which we call-- define in terms of the standard [INAUDIBLE] And then the alpha minus m i, alpha m i, so it would be equal to m N m i. OK? So this is oscillator number for the m's mode and in i directions. OK? So in this equation there's no summation of i. Also there's no summation of m, just a-- OK? So I hope this is clear. Good. Any questions?
So now let's look at those equations. Now let's look at the-- those mass-shell conditions. So the quantum [? variable. ?] OK?
AUDIENCE: Excuse me. What does operators x and p do in those vacuums?
PROFESSOR: Hmm?
AUDIENCE: Operators x and p. What do they do with those right here?
PROFESSOR: No no no. So we take-- so back here, so we take the vacuum to be a [INAUDIBLE] state of p. And then yeah, then that's it. Yeah.
AUDIENCE: [INAUDIBLE] p i [INAUDIBLE] right here, what do they do?
PROFESSOR: So this is [INAUDIBLE] state of p. And the action of x on p, it just asks what do you do in quantum mechanics. Yeah. Yes?
AUDIENCE: [INAUDIBLE] kind of a dumb thing, but. So the p mu, that only includes the i [INAUDIBLE], or all?
PROFESSOR: All components. All components. But then they must be satisfied.
AUDIENCE: Right, right. So they have to satisfy--
PROFESSOR: Then they have to satisfy this kind of constraint.
AUDIENCE: I see.
PROFESSOR: Other questions? Good. Good.
So now, we can write down-- so now let's write down the mass-shell condition in terms of-- so this is a classical equation. OK? These are the classical equations. So now we can write down the quantum version of it. So we can write down the quantum version of it.
So each state, so the typical state, here, will carry some p, will carry some p. So p i are independent, but then p minus p i and p plus, for example, yeah, for x plus, this will also give you a p plus. For p i and p plus are independent. But then the p minus, then it's determined from those equations, determined from these mass-shell conditions. OK? So now let's write down those mass-shell conditions in terms of quantum form. So now let's, again, look at the mass-shell conditions.
So let's first, again, do the open string. So we just take these. So when you go to quantum, then you have to worry about the ordering. OK? Then you have to worry about the ordering, because now they don't [? commute. ?] OK? So now you have-- about the ordering. So let me-- for the moment, don't-- then there's an ordering constant, et cetera. And it's easy to understand what should be the ordering constant, because this is a string, and each oscillation mode behaves like an-- it's just a harmonic oscillator. And then you just add up all the zero-point energy of the harmonic oscillator, and that will give you the ordering constant. OK? It's just the same as a harmonic oscillator problem.
So to translate this into a quantum operator expression, so I just do it-- [? isolate ?] the harmonic oscillator. OK? Because I just-- have essentially have m harmonic oscillators. Have all these different harmonic oscillators. Then this is easy. So I just copy this one of alpha prime. So this is m from minus infinity to plus infinity. So let me rewrite it from m equal to 1, to plus infinity. Then I have a factor of 2. Then the overall factor becomes 1 over i, 1 over alpha prime. OK?
And now, let me-- also making the sum over i explicit, so from 2 to D minus 1, all the transverse directions, and then I sum over m equal to 1 to infinity. And this, I have written down before. This is essentially just this m times the oscillation number. m N m i. OK?
So now I have to write down the zero-point energy. So let me call it a zero. So the zero-point energy, so you just do it as a harmonic oscillator. So this is for the oscillator number. So for harmonic oscillator, we have m plus 1/2, OK? So for each oscillator, you have 1/2. So a zero must be equal to 1 over alpha prime sum over i sum over m then 1/2 times m. OK? So is this clear to you? Yes?
AUDIENCE: So in here, we're not actually summing over i, are we? Is there an implicit sum over i?
PROFESSOR: Oh yeah, oh, sorry. Here. Here, repeated index always means we summed.
AUDIENCE: Even though they're both up indices?
PROFESSOR: Yeah, yeah. Yeah, because we are working with Minkowski metric.
AUDIENCE: I see. OK.
PROFESSOR: OK? So essentially, you have the harmonic oscillator with a frequency m. OK? Because that's what each the-- yeah, I should have emphasized one step. Let me see. Yeah, I did not write it down explicitly here. But if you remind yourself, when you quantize a free quantum fields theory, the harmon-- now I have erased my harmonic expansion-- and in the harmonic expansion, under the nth mode, we have frequency n. OK? Yeah, say-- let me just add it here. So when we write down [? pi-- ?] say tau minus sigma-- yeah, it'll be some pre-factor.
So this n, this just the frequency of each mode, OK, as a harmonic oscillator. So that's why, here, for the zero point [INAUDIBLE], so each one, each mode, contribute 1/2 m. OK? So is this clear to you?
AUDIENCE: And you can also get that from the computation relationship?
PROFESSOR: Yeah.
AUDIENCE: [INAUDIBLE].
PROFESSOR: Yeah, let me just say, I want to write down the quantum version of this equation. The way I do it is that I write down the quantum version of the harmonic oscillator. Treat each mode as a harmonic oscillator, and then the zero-- then this will be just a sum of an infinite number of harmonic oscillators. And each harmonic oscillator have a frequency n. So each harmonic oscillator have frequency n. OK? So each harmonic oscillator has a frequency n. Then this is the oscillator number times the frequency. And the zero mode is just 1/2 times the frequency. Yeah. Clear? Good.
And similarly, we can write down the equation for the closed string. Again, sum over i sum over m not equal to zero, or sum m equal to one to infinity, then m, m N i plus m N m i tilde. OK? And then, again, plus a zero. So this here, a zero, it's essentially the same. Is the-- so now you have this equation. So this is alpha 2 sum over i sum over m from minus 1 to infinity, from 1 to infinity, 1/2 m plus m. So 1/2 m plus m because there's two modes here. OK? Yeah, maybe I should write m 1/2 plus 1/2. Yeah?
AUDIENCE: I'm still confused about something. So where exactly did the a zero come from? Where is it defined before?
PROFESSOR: No no no. No, a zero come from here, come from I'm rewriting this equation as a quantum equation. So this is-- so if you look at it here, here, each one is a dagger and a. So there's an ordering issue here. And there's an ordering issue here. So I'm just giving you a simple trick to find out what is the-- how to resolve the ordering issue, because each term is like a harmonic oscillator. And then this will be just like, exactly like a harmonic oscillator.
AUDIENCE: OK. So you're just taking all those contributions and packaging it?
PROFESSOR: Yeah, that's right, that's right. Yeah, just like you're writing down the energy of a harmonic oscillator.
AUDIENCE: Gotcha. OK.
PROFESSOR: Yes?
AUDIENCE: I'm a bit surprised that these terms contribute to m squared, not to m. They look like [INAUDIBLE] of a--
PROFESSOR: Which one?
AUDIENCE: I mean [INAUDIBLE] so the a zero, all zero-point energies? Intuitively I would have thought that they would add up to-- they would contribute to the mass of the string, not to the mass squared.
PROFESSOR: It depends on where is your intuition come from.
AUDIENCE: I don't know, it-- is it--
PROFESSOR: Yeah, this is a-- this is in the-- yeah. I think, again, so, so here, I'm assuming the-- yeah, let me just-- I understand where you've-- yeah, let me just explain one more thing. Let me just explain one more thing. So that equation, which I write in that form, m square equal to that thing. OK?
And this should be understood as the 2 p plus p minus-- minus p i square, say minus-- yeah, minus p i squared equal to that thing. OK? And then this can also-- so this essentially gives you p minus. You could do this, the rest. And the p minus is the image in the light-cone frame. And so, you are just [? conceding ?] the image, but in the light-cone frame.
AUDIENCE: Why does that get p minus, sorry?
PROFESSOR: Yeah. I'm just saying this equation itself-- think about how we derived this equation. This come from here. Come from here. Yeah, I'm doing it a little bit fast today. So come from here. So this equation should be considered as a constrained equation which you solve for p minus. And p minus is precisely the image in the light-cone frame. Yeah, this is a precise image in the light-cone frame. Yeah. That's why. Yeah. So is it clear?
AUDIENCE: I need to think about it a little bit.
PROFESSOR: Yeah. And so, that's, yeah. Yeah. This is a good question. I should have emphasized this point a little bit earlier, in jump from here to there. Yeah. You should think of this-- so this is an equation which you can imagine it as solve for p minus. And p minus is the image in the light-cone frame. And then all these contributions, the right-hand side, can be considered as the contribution to the light-cone image. And then we're just repackaging it in terms of the mass term. Yeah.
So now we have a problem. Because if you look at the zero-point energy-- so any other questions?
AUDIENCE: So the mass square, the mass of the string [INAUDIBLE].
PROFESSOR: The mass over the-- no no no no. That's not the way you should think about-- sorry, say it again?
AUDIENCE: Because you said p minus the energy, and then.
PROFESSOR: p minus is the light-cone energy.
AUDIENCE: Light-cone, yeah.
PROFESSOR: Yeah. So the light-cone image-- yeah, the light-cone energy. Yeah, this is a light-cone [? image. ?] Yes?
AUDIENCE: But then is-- what he was asking is why the contribution from the zero point energy is-- you are contributing to the m squared, right? So.
PROFESSOR: Yeah. My answer is that the zero-point energy is contributing to the light-cone image. It's contributing to the light-cone image. Yeah, just repackage it in terms of the mass-squared term.
AUDIENCE: One more comment. That [INAUDIBLE] in the [INAUDIBLE] case and that you will find the [INAUDIBLE] p minus. That is [INAUDIBLE] interpretation.
PROFESSOR: Yeah. Just say because we are going to the light cone, and in the light cone, the p minus is the image. And then we repackage it into the mass-squared term. Is it clear?
Yeah, so what he was asking is-- so his intuition is right, because this-- at least in the light-cone gauge, you can interpret it-- so this zero-point image is contributing to something like image, rather than something like mass square, even though this equation is acting like a mass square. Yeah. That was my explanation to him. Yeah?
AUDIENCE: So one other question. If indeed there is an infinite constant [INAUDIBLE], like there is normal quantum physics, how does string theory get around this issue of-- so it seems that you're still looking at this issue with infinite energy in a vacuum.
PROFESSOR: We're not going into that. Yeah, we're not going into that.
So this is a standard issue. So now we see that you have-- so now you see you have a sum of an infinite number of modes, and each mode contributing to 1/2 m. So each mode contribute to 1/2 m. So this is apparently an infinite answer. OK? But now we have a trick. But now we have a trick. The trick is this sum is actually equal to--
AUDIENCE: Oh my gosh.
[GIGGLING]
PROFESSOR: So we have a trick. And the trick is that this is actually-- we equate it to minus 1/2-- minus 1/12. OK? So there are many ways to justify this thing. But I will not do it here. I will only do it in one way. One way, which is the quickest way, but maybe it's most unsatisfying way physically. But it's the-- mathematically, the quickest way. OK? And this is so-called zeta function regularization.
So typically-- so a trick we often used in physics is that when you go to-- so when you get an infinite quantity, what you do is you slightly change the form of your quantity so that this is finite. And then you manage to take the limit. To go back to the original limit. And yeah, let me first just write down the answer for you. And then I talk about philosophy.
[LAUGHTER]
PROFESSOR: So the key is a [INAUDIBLE]. And let's define a function called the zeta function. So this is the so-called Riemann zeta function. And so this is sum of n equal to 1 to infinity 1 over n to the power s. OK? So as you all know, this sum is only convergent only for s greater than 1. And it's a famous sum that when s equal to 1, this is a logarithm divergent. OK?
So this function have [? a pole. ?] So [? this ?] thing over these [? a ?] function. So what you can do is you can do the sum. For s greater than 1, then you can actually-- we'll call the analytic function letter s. Use this as a definition for this function. And this function is well defined for s greater than 1. And then you find this function has a pole.
And the zeta equal to, say, 1. At s, you could do 1. Because 1 into this-- as a logarithm divergence, yeah, for 1 plus 1/2, et cetera.
But this function-- or, I can do it here-- but this function have an analytical condition. But this function, this analytic function, can be analytically continued beyond s equal to 1 to smaller value of s. In particular, you find can be analytically continued to minus 1.
So minus 1 is a situation, what we are seeing-- looking at here, with s equal to minus 1, this equal to that. And then this function when you [INAUDIBLE] to minus 1, you find it's actually finite, and then given by minus 1/12. OK? So this is a rationale for the manipulation.
So this is very similar-- this is very similar to dimensional regularization you used in quantum field theory. And you see, certain integrals are divergent. Say, certain integrals are divergent in four dimensions. And then, the trick we did, when you do your quantum field theory, is that we promote the dimension as a variable. Rather than equal to 4, we called it general d. And then, for that general d, then there exists some range of that d, which is that integral is finite. And then you can evaluate it as an analytic function of d. And [? analytic ?] continue to d equal to 4.
And sometimes that just give you a finite answer. And sometimes that still gives you a divergent answer, and then you need to do [? regularization. ?] But sometimes when you do that trick, you just get a finite answer, and then there's even no need to do a [? regularization. ?] That trick just works. So this is exactly the same as that trick. So this trick is exactly in the same sense as that.
But of course, this is only a mathematical trick. And to justify it physically, you have to think about the-- to justify it physically, it's the same as the philosophy for your [INAUDIBLE] in general quantum field theories. It's that this system have many, many high-energy modes. So the divergence come from very large m, have very, very high-energy modes. And so the justification is that-- so those high-energy modes, so the low-energy physics, which is related to the zero point [? energy ?] [? excitement, ?] should be insensitive to the physics of those high-energy modes. Then you can subtract the infinity, et cetera. So that philosophy's the same as what you normally do in the [? regularization ?] quantum fields theory. OK. Any other questions here?
AUDIENCE: So can we say that the true physical theory doesn't have any divergences, but it's just this effective theory that kind of works out, has divergences that we then, kind of, get rid of by the strings? But the real physical theory, that real wouldn't, shouldn't wouldn't have any divergences?
PROFESSOR: So you just say the physics-- say this particular quantity does not depend on the details of your UV physics. So this divergence itself comes from a particular assumption of the UV physics. But you can modify your UV physics in a certain way, and this particular answer should be independent of those details of that UV physics. Yeah. So this is a standard philosophy behind the regularization.
And so you can justify this answer using many different ways. You can also justify it-- so you can also just put some constant in there, and then impose some other self-consistency conditions, and you can determine this answer. Anyway, there are many ways you can derive this answer. And this is one way, or the one quickest way of doing it, so we are just do it here. So any other questions?
Good. OK. So now, from here, we can find that for the open string, the a zero is just equal to minus, d minus 2 divided by 24, 1 over alpha prime, for the open. And then for the closed string, is equal to minus d minus 2 divided by 24 alpha prime over 4. Closed. OK?
Free Downloads
Video
- iTunes U (MP4 - 183MB)
- Internet Archive (MP4 - 183MB)
Subtitle
- English - US (SRT)