Flash and JavaScript are required for this feature.
Download the video from iTunes U or the Internet Archive.
Description: This session covers fabrication, microstructure and mechanical properties of osteochondral scaffold.
Instructor: Lorna Gibson
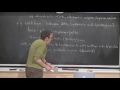
Lecture 13: Tissue Engineer...
NARRATOR: The following content is provided under a Creative Commons license. Your support will help MIT OpenCourseWare continue to offer high-quality educational resources for free. To make a donation or view additional materials from hundreds of MIT courses, visit MIT OpenCourseWare at ocw.mit.edu.
LORNA GIBSON: So last time, we finished talking about trabecular bone. And what I wanted to talk about this week was tissue engineering scaffolds.
So the idea with tissue engineering is you want to be able to repair damaged or diseased tissue. And typically, that's done by regenerating the tissue in some way. So in your body, many types of cells, like maybe not blood cells, but most types of cells for sort of structural tissues are attached to an extracellular matrix. And this is sort of a schematic of the extracellular matrix here. And the composition depends on the type of tissue that's involved.
For example, in skin, it would be collagen and something called glycosaminoglycans and elastin.
In bone, it would be collagen and a mineral-- a calcium phosphate mineral, hydroxyapatite. So the composition varies. But the idea with the tissue engineering scaffold is that you want to make a material that essentially substitutes for the extracellular matrix. And it does so on a sort of temporary basis.
So the idea is you put something in the body. The cells attach to that, whatever scaffold you put in. And the scaffold has to be made in such a way that the material-- that the cells, they can migrate through it. They can attach to it. They can differentiate. They can proliferate. So the cells can do all their normal function.
And the idea is that as the cells are doing their normal function, they then secrete the natural extracellular matrix. And the engineered thing that you put in is resorbed. So there has to be a balance between the rate at which the scaffold you've made resorbs and the rate at which the cells are depositing the new native extracellular matrix. So that's one of the key things about this.
So this is just an example of sort of a schematic of an extracellular matrix. In this case here, there's collagen fibers. So these guys here collagen fibers. And these kind of hairy-looking things are proteoglycans. So they have a core of protein with sugars kind of hanging off of them. And there's different kinds of GAGs, they're called, that hang off of them. So one's chondroitin sulfate. The CS here stands for Chondroitin Sulfate. There's one called dermatan sulfate. And there's one called heparin sulfate. So there's different of these glycosaminoglycans. So let me write down some of this, and then we'll kind of get into this.
So what I wanted to do today was show you some examples of tissue engineering scaffolds. Show you some of the sort of design requirements. So you have to have, obviously, a material that's porous so the cells can get in there. And that's where the cellular solids comes in. So we'll talk about some scaffolds, some of the sort of design requirements for them. And also, we'll talk a little bit about processing of the scaffolds and mechanical properties of the scaffolds. So I'm hoping I can finish most of that today.
And then next time, I have a little case study on osteochondral scaffolds. So osteo means bone. And the chondral means cartilage. So this was sort of a two-layer scaffold that we developed in collaboration with some other people at MIT and some people at Cambridge University. And it went from a research thing to a startup thing and being used clinically. So I was going to talk about that Wednesday. OK. So let me just get started here.
So the goal of tissue engineering is to regenerate diseased or damaged tissues. And in the body, the cells attach to the extracellular matrix. And that's sometimes called the ECM. Sometimes, the scaffolds are called scaffolds. And sometimes, they're called matrix because the extracellular matrix is called matrix.
So then, the composition of the ECM depends on the tissue, but it usually involves some sort of structural protein. So something like collagen or elastin. It also typically involves some sort adhesive proteins. So something like fibronectin or laminin. And it involves these proteoglycans, which are the core of protein with a sugar hanging off them. Whoops.
And the sugars are typically glycosaminoglycans. And for short, people call them GAGs, just because it's easier to say.
So some examples of the GAGs are chondroitin sulfate. And we're going to talk more about that a little later because that's one that we've used in making collagen-based scaffolds with [INAUDIBLE].
So for example, if you look at the composition of extracellular matrix in something like cartilage, it has a collagen component and a hyaluronic acid component and some GAGs. And this hyaluronic acid is a proteoglycan.
If you look at bone, extracellular matrix in bone, it's made up mostly of collagen and hydroxyapatite.
And if you look at skin, skin is made up of collagen, elastin, and proteoglycans. And the idea is that the cells have to be attached to this extracellular matrix in order to function. Or, they have to be attached to this or to other cells in most cases.
So next week, I'm going to talk a bit about cell mechanics. And we have some video where we watch a cell deforming a scaffold. And I don't have videos to show you, but I had a student who took videos of cells migrating along with scaffolds. We'll look at how the stiffness of the scaffold affects how the cells migrate along the scaffold. So that's kind of the native ECM in the body.
And the idea with tissue engineering is that you want to make a scaffold that's porous that mimics the extracellular matrix in the body. So, say, there's some tissue that's damaged. Say there's damaged cartilage. You want to provide sort of an extracellular matrix that's a sort of synthetic thing that's going to provide the same function as the ECM in the native tissue.
So people have been working on scaffolds for regenerating all sorts of different tissues. And probably, the most successful one has been used to regenerate skin. And there's been scaffolds available for regenerating skin for probably almost 20 years now. And one of the first ones was developed by Professor Yannas in mechanical engineering here at MIT. And it's actually still sold by a company called Integra.
But this research to develop scaffolds for lots of different tissues, orthopedic tissues, things like bone and cartilage, cardiovascular tissues, nerve-- like Professor Yannas works on peripheral nerve these days. People have looked at trying to make scaffolds for gastrointestinal tissues. So all sorts of different tissues.
And at MIT, there's quite a lot of interest in this. There's a lot of people working on it. So Bob Langer works on it. Linda Griffith. Sangeeta Bhatia. There's really quite a number of people at MIT who work on it. Those are just some of the people at MIT.
So the idea is in the body, the cells are constantly resorbing the extracellular matrix and depositing more. So if you think about bone, for example. Remember we said bone grows in response to load? Even in healthy bone, the bone is constantly being resorbed and deposited.
And in normal bone, the rate of resorption and the rate of deposition is roughly the same. When people get osteoporosis, the thing that happens is that that balance gets out of whack. And so it's not being deposited at the same rate it's being resorbed.
And the idea with the tissue engineering scaffolds is that they degrade over time. And that the cells that were attached to them are forming their own extracellular matrix. So there's kind of a balancing act between the cells depositing the native ECM and the tissue engineering scaffold that was provided, say, by the clinician being resorbed. So the scaffolds are actually designed to degrade. And controlling that degradation rate is one of the design parameters of the scaffolds.
OK. So that's kind of the overall, kind of big picture. And what I wanted to talk about next was some design requirements for the scaffolds.
So if you think about it, there's different sort of ways you can think about what the requirements are. So you have to make the scaffold out of some solid. And there's some requirements for the solid. So obviously, you want a solid that's biocompatible. That's one kind of main requirement.
Another requirement is that not only the solid has to be biocompatible, but when the solid decomposes, if it decomposes into other components, they have to be biocompatible too. So you don't want the solid to degrade during this resorption process into toxic components. That would be a bad idea.
And then, the other thing is the solid itself has to promote cell attachment, and cell proliferation, and cell migration, all these kinds of things. So we're going to talk about some different materials for the scaffolds. And some of them are sort of native proteins. So some of them are things like collagen. Collagen already has binding sites for cells to attach to it. Obviously, it's one of the proteins in the native ECM.
There's also a number of synthetic polymers you can use. And with the synthetic polymers, they don't have natural binding sites for the cells. And so you have to coat them with something else. So you have to coat them with, say, adhesive proteins so that the cells will attach to them.
So we're going to talk about the requirements for the solid. Then, you make the solid into some sort of porous, foamy thing. I think I have some slides here.
Here's an example of a collagen GAG scaffold. And this is one of the ones that is made in Yannas' lab. And you can see it looks a lot like a foam. It's very, very porous. And there's some requirements for the sort of cellular structure, the foamy structure of the scaffold as well.
So typically, you want interconnected pores so it's easy for the cells to migrate in. Typically, you want pores to be within a certain range. It turns out if the pores are too small, it makes it difficult for the cells to get in. Sometimes, they can by eating away at the material. But typically, the pores-- you want them to be bigger than a certain size.
You also want them to be smaller than a certain size because how much specific surface area, how much surface area per unit volume you've got, depends on the pore size. The smaller the pores, the more surface area per unit volume you have. And then, the number of binding sites you have for cells to attach to it depends on that specific surface area. I'm going to write all this down, so I'll do that.
So there's requirements for sort of the pore structure. And then, there's also some requirements for the whole scaffold itself. So for instance, it's got to have some minimal mechanical integrity. So there's sort of some requirements for that. So there's requirements for the solid. There's requirements for the sort of cellular structure, the porous structure. And there's requirements for the overall scaffold. So let me write some of these things down.
So there's some requirements for the solid. So it must be biocompatible. It must also promote cell attachment and proliferation in the cell functions. And then it must degrade into nontoxic components.
So there's some requirements for the cellular structure, too. And what you want to have is a large volume fraction of interconnected pores. And so you want that to facilitate the cell migration. And also, the transport of nutrients into the cells.
So you also want the pore size to be within a critical range. So you need the pores bigger than a lower limit so the cells can migrate through easily, can kind of get in there. And you want the pore size to be less than an upper limit to have enough surface area to have enough binding sites to actually attach cells. And for different tissues, there's different critical ranges of the pore size.
So for example, for skin they found that you want to have a pore size between about 20 microns and about 150 microns.
And for bone, the pore sizes that people tend to use are between about 100 and 500 microns. So there's the pore size.
And one other feature is that the pore geometry should be conducive for the cell type. So lots of cells are somewhat equiaxed. Maybe a little elongated.
But if you look at something like nerve cells, like peripheral nerve, they're incredibly elongated. So you want to have pores in the scaffold that are also very elongated.
And then for the overall scaffold, it needs to have some mechanical integrity. You guys OK? Yeah? We're good.
Oh, achy. Yeah. I know.
So you want to have some overall mechanical integrity. I mean, the thing has to be put into the body in surgery. And people are going to be pushing and poking at it. And so it has to have some just overall mechanical integrity.
Also, it turns out that if you put stem cells into scaffolds, the types of cells they differentiate into depends in part on the stiffness of the scaffold. So you want to be able to control the stiffness of the scaffold.
AUDIENCE: How do they do this research? Do they use animals? Or they do it in vitro?
LORNA GIBSON: Yeah. So the question is, how do they do the research? So they do a sort of series of different things.
So at one level, you could have the scaffold and you'd put cells on it. Say you're making a bone scaffold. You'd put osteocytes onto it. So one level, you just put cells onto it. And you want to see, are the cells attaching? Are they dying? Are they proliferating?
So sometimes, what people will do is put the-- they'll seed a certain number of cells at a certain time. Say, time 0. Then, they'll look at how many cells are attached at 24 hours or 48 hours. And you kind of see the cell attachment. You can measure relatively easily.
Another thing people do is animal studies. So for instance, Yannas does research on peripheral nerves and scaffolds for peripheral nerves. And they cut a piece out of the sciatic nerve of rats. So obviously, they have a surgeon and do a surgery thing with it. You can't just kind of do this in the lab. You've got to get permissions and stuff to do it.
And then, they put in the scaffold. And the scaffold's actually in a tube. And so they put the two stumps of the nerve end at either end of the tube, and then the tube's filled with a sort of porous scaffold that we're talking about here. And then, they wait some period of time. And they take video of rat running, things like that. They then sacrifice the rat and they do histology. And they look at the sort of cross-sections and see what it looks like.
And so I'm going to talk next time about this osteochondral scaffold we worked on. So we did cell studies. We did goat studies. We put into goat knees. There was a longer term sheep study. And then, the student who's in Cambridge, England, started up a company and he ended up getting approval in Europe to start clinical trials. And then he worked with an orthopedic surgeon who started putting it in people. But typically, they're looking at cells, looking at animals before you get to the people stage.
And one of the things people do when they're making these scaffolds is you want to use materials that already have some sort of regulatory approval. So say FDA approval or approval in Europe. So typically, people don't start with a brand new material from scratch. Because to get approval for that would just take a very long time. So typically, people start with-- the solid material is already approved for some other sort of use. OK.
So one requirement for the overall scaffold is it has to have sufficient mechanical integrity. Sufficient.
And then also, as I mentioned, the stiffness of the scaffold can affect differentiation of cells. And the other thing that is really a factor for the overall scaffold is you want to control the rate of degradation of the overall scaffold. So you want that rate to be matched to the rate at which the new tissue is forming. So it has to degrade at a controllable rate.
OK.
So I want to talk about the materials that people use. And you can kind of break them down into a few classes. So one class is natural polymers. So things like collage. So you can get collagen. And that's an example up there of a scaffold that's made with collagen.
Another class of materials is synthetic biomaterials. And if you've had stitches or surgery, you may know that some of the sutures they use are resorbable. So some of those polymers that they use for resorbable sutures are also used for tissue engineering scaffold.
And then, there's also hydrogels that people use as well. So those are probably the three main groups are sort of natural polymers, synthetic biopolymers, and I guess the hydrogels are sort of a subset of the sympathetic biopolymers.
So collagen is probably the most common kind of natural polymer that's used. They also use GAGs. And this scaffold up here is made by making a coprecipitative collagen with a GAG chondroitin sulfate.
People also use alginate. I think one of the project groups-- you guys are going to make some sort of alginate scaffold, right? No? [INAUDIBLE] foamy thing? Yeah.
And people also use something called chitosan, which is a derivative of chiton, which is what's in the exoskeleton of insects and things like lobsters. So those would be all examples of natural polymers that can be used and people have tried.
I'm going to talk a bit more about collagen, just because it's the most common one. So collagen is a major component in the natural extracellular matrix. And not surprisingly, it has binding sites for cells to attach to it. So if you use that, that kind of takes care of that issue.
Let me put it down here. So collagen exists in many types of tissues. Exists in skin. Exists in bone, cartilage, ligament, tendon-- cartilage. So it's very common. It has surface binding sites for cells.
It has a relatively low Young's modulus. So the Young's modulus is a little less than a gigapascal.
But you can increase the modulus by either cross-linking or by using it in conjunction with some synthetic polymers.
And I'm going to talk a little bit about how you make these scaffolds up here. And the first step in making those scaffolds is you put the collagen in acetic acid, and then you add the glycosaminoglycan and it forms a coprecipitate.
And the fact that it forms a coprecipitate with the glycosaminoglycan, the GAG, means that you can use a freeze-drying process. And that's how that's made.
Collagen is one option. So then synthetic biopolymers is another option. And as I said, typically they use the materials that are used for resorbable sutures. So there's several of those.
There's something called PGA. That's polyglycolic acid. And something called PLA. That's polylactic acid. And then you can combine those two and make something called PLGA polylactic co-glycolic acid.
And you can control the degradation rate of these things by controlling the molecular weight. And in this case, you can also control it by controlling how much of each of those things you put in.
And there's another one called polycaprolactone. So those are several synthetic biopolymers that people use. There's lots of different materials, but these are just some typical ones.
And then another class are hydrogels, which are produced by cross-linking water soluble polymers to form an insoluble network. And those are typically used for soft tissues. Sometimes, they're used for things like cartilage.
And again, there's a few different materials that are commonly used. One's PEG, Polyethylene Glycol. One's PVA, Polyvinyl Alcohol. And another one's PAA, Polyacrylic Acid. So for these synthetic polymers, there's many different processing techniques available. And I'll talk a little bit about some of the processing techniques. But one of the limitations is they don't have natural binding sites. And you have to coat them with some sort of binding agent, like an adhesive protein, to get the cells to attach to them.
And then, as I mentioned before, you have to make sure that whatever material you choose, if it's a synthetic material that when it degrades, it's not toxic to the cells. Because you don't want to have some sort of toxic reaction or inflammation. OK. So there's a couple more things about materials. So those are all polymer-based materials.
When people are trying to make scaffolds for bone tissue engineering, they also include a calcium phosphate mineral. And there's different versions of the calcium phosphate. So they can include-- you can buy, for instance, hydroxy powders now. There's another calcium phosphate called octacalcium phosphate, which will, with water, turn into hydroxyapatite. So typically, there is this mineral, some sort of calcium phosphate, is combined with either collagen or with one of these synthetic biopolymers.
And one other option is something called an acellular scaffold. And what that is they take some natural tissue and they remove all the cell material from it. And so when they remove all the cell material, what they're left with is the native ECM. And that's called an acellular scaffold. So it's a native ECM with all the cell matter removed.
And they remove the cells by-- they can use sort of a physical agitation or chemical, or using enzymatic methods. Using something like trypsin to get rid of the cell. So there's ways that they can get rid of the cells. OK. So are we good so far? So there are some requirements for what materials we kind of use, what the cell structure should be, and these are some examples of typical materials.
So I wanted to talk a little bit about the processing of the materials. Let me wait until people catch up a little.
Oh, and I have some scaffolds I was going to pass around. So this big sheet is a piece of the collagen GAG scaffold that I showed a minute ago. And then this little piece is a mineralized version of that. So this has the collagen plus calcium phosphate plus hydroxyapatite in it. OK.
So this slide shows some examples of different scaffolds that people have made. And I was going to talk a little bit about some of these methods. And why don't I talk about them, and then I'll write some notes on the board.
So this top one here on the top left, that's the collagen GAG scaffold that's made in Yannas' group. And that's made by a freeze-drying process. So you put the collagen in acetic acid, then you put in the GAG. The GAG and the collagen form a coprecipitate. And then you can freeze that.
And if you freeze it, what happens is-- it's just like if you freeze saltwater. The water freezes. The pure water freezes. And you've got increasingly higher brine content in the bit in between the water grains, or in between the ice. So you get the sort of solid ice forming. And the collagen and the GAG are kind of squeezed into the interstitial bits between the ice crystals.
And then if you sublimate the ice off, you're left with this porous kind of structure that looks like a foam. So that's made by a freeze-drying process. And I'll go over it in a bit more detail when I write the notes on the board.
You could also foam some of these polymers. So just blowing a gas. The same way you can blow a gas through engineering foam. You do the same thing with some of these polymers.
This one's made by foaming.
You can have a fugitive phase process. So this is made by salt leaching, the second row on the left there.
So you could imagine you could take a polymer powder. You could mix it with salt. You can sort of mix them up, combine them together. You heat it up to get the polymer to melt and to sort of form a connected mass. And then you leech out the salt. And then you get pores where the salt was.
This one here is made by an electrospinning process. So you have a nozzle. You feed the polymer through the nozzle. Then you have plates that are charged and you get fibers forming and kind of scattered in different directions by the electrospinning process.
Then, this one here represents scaffolds that are made by things like 3D printing, selective laser centering. You can have laser-sensitive polymer. And you can produce scaffolds that way.
I think the geometry of this one matches some part in the body. I think it was a knee or something like that.
And then, these two examples down here are the acellular scaffolds. That's what I was talking about at the very end there. So those are from porcine pork heart tissue. You know, pig heart tissue. And those are mostly elastin. And they've had all the cell matter removed from them. OK.
So you can kind of see that these synthetic scaffolds here have a structure that's not so different from these native ECM scaffolds down here. OK. So let me write some of the things about the processing on the board. Let me rub this off. Start over here.
So these freeze-dried scaffolds are used for skin regeneration. And I think I have some more slides here.
So it's kind of a two-step process. In the first step, you make what they call a slurry. So you make the slurry by taking the collagen. And for skin, I think you want type 1 collagen. Yeah, skin is type 1 collagen. There's different types.
You put it in acetic acid, and then you add the GAG. And we use chondroitin 6-sulfate is just the particular GAG that we use.
And one of the things that the acid does is that it swells the collagen. And collagen has a sort of periodic structure in it, sort of periodic banding. And the acid destroys that periodic banding structure. And that helps increase the resistance to having some host immune response. So that you remove the immunological markers and it makes it less likely that the scaffold's going to get rejected by the body.
So then when you put the GAG in, you form a coprecipitate. So this next step just shows kind of mixing the whole thing up.
And then you've got kind of a little slurry that you can store. And then, this is the freeze-drying step here. So you put the slurry, the suspension, into a pan. Kind of just like a cookie sheet, really. And then you freeze it.
So if you think of this phase diagram here, where you have temperature and pressure. So here we have liquid, solid, and vapor.
So if you start off at this point here, you freeze it. So you've reduced the temperature. So that forms the ice. And the ice is surrounded by the collagen and the GAG fibers.
And then if you do the sublimation step, you reduce the pressure and increase the temperature a bit. And then you get over to the vapor end of the world. And then you're left with this porous scaffold.
And then, let me see. Let me do one more step here. And then you can control the size of the pores by controlling the freezing temperature. So the size of the pores is exactly related to the size of the ice grains that are forming. And the faster it freezes, the smaller the grains are going to be. And then, the smaller your pore size are going to be. So you can control that.
The type 1 collagen is mixed with acetic acid. And it then swells the collagen and disrupts periodic banding. And it removes immunological markers.
And then you add the GAG, the chondroitin 6-sulfate. And then that cross links with the collagen and forms a coprecipitate. And then you can freeze dry that to get the porous scaffold.
And typically, the relative densities of these scaffolds is very low. So typically, they're 0.5% dense. The relative density is 0.005. So they're 99.5% air and the rest of it's the collagen and the GAG. And the pore sizes are typically between about 100 and 150 microns.
And Yannas uses the same scaffolds for the nerve regeneration. And he uses a directional cooling. And that then elongates the pores, so that-- the idea is that they elongate so the nerves kind of grow along that length. So that's one way.
Another way is leaching a fugitive phase. So let's see. I think-- yep. Here we go. Back to there.
So if you look at the one on the second row on the left, that's done by using salt as the fugitive phase. People use other things. You can use wax. Paraffin wax works as well. So it doesn't have to be salt. There's different things you can use.
So you combine a powder of the polymer with your fugitive phase Say, salt. Then, you heat it up to get the polymer to bind. And then you leach out the salt. So you can control the porosity by the volume fraction of the fugitive phase, and then the pore size by the size of whatever the fugitive phase is.
Another technique is electrospinning. The idea is you produce fibers from a polymer solution that you extrude through a nozzle. And then you apply a voltage across some plates to spin the fibers. And then you get a network of these fibers. And typically, their micron-scale diameter.
And the last method I'm going to talk about is rapid prototyping. So you can think of using 3D printing. Or you could use selective laser centering or stereo lithography using a photo-sensitive polymer. So the idea is-- you know how this works. You just build up layers of solid, one layer at a time.
And then you can make complex geometries with that. So that's one of the advantages. If you wanted to make a part to fit a particular place in the tissue, then it's convenient that you can control the geometry of the whole part.
OK. So that just kind of summarizes very briefly, kind of how the tissue engineering scaffolds are meant to work, what kinds of materials people make them from, and a few of the processes. And there's many, many processes. These are just sort of a few common processes.
I wanted to talk a little bit about the mechanical behavior because that's kind of what I do. And so this next plot just shows a stress strain curve in compression for a collagen GAG scaffold. And I'm hoping that by now you're getting the idea all of these cellular materials have this kind of shape of a curve. So there's the same kind of linear elastic regime, and then a collapse plateau. These collagen things, as you can imagine just pressing them in your hand, they fail by buckling, by inelastic buckling. And then there's a densification regime. So they look like all the other kinds of curves that we've got.
One of the things we've done in the modeling is typically in the model, we want to be able to calculate or measure the modulus of the solid or the strength of the solid from which the thing's made. So [? Brendan ?] Harley was one of my PhD students. And he took a little microscope, cut a little strut. The struts are very small. The pore size is 100 microns. So the struts are on that order.
He glued one end of the strut to a glass slide, and then he used an AFM probe to do a little bending test on that little strut. If he could measure the deflection, he knew the length. He knew the geometry of the strut. He could figure out what the modulus was for the solid. So he backs out what the modulus is.
So he did these measurements on a dry strut. And then by comparing the overall modulus of a dry scaffold with a wet scaffold, he estimated what the modulus of the wet scaffold or the wet strut would be. So the modulus of the dry collagen GAG was 672 megapascals. A little less than a gigapascal. And wet it was about 5. So there's a huge difference between the wet and the dry. OK. So let me just write a few notes about that.
So in compression, there's the three regimes that we see for all these cellular materials. And so you can estimate the modulus by using the model we have for the foam for the modulus. The modulus of the foam divided by the modulus of the solid goes as the relative density squared. And that's related to bending in the cell wall.
And then, the collapse plateau is related to elastic buckling. And so that's equal to some constant times E of the solid times the relative density squared. And that's related to elastic buckling.
And then we measured E of the solid doing this little AFM beam bending test. OK.
And for one of these low-density scaffolds, we measured the modulus. We measured the buckling strength. And we got pretty good agreement by using these equations here. And the good agreement was if this constant was 0.2. That was the strain, that buckling. Yeah.
AUDIENCE: So what does it mean to be wet in here? And why is it so much lower?
LORNA GIBSON: Well, we make the scaffolds by this freeze-drying thing. And like this thing I passed around, that was dry. We just immerse it in water. We just put it in water. And then, he does the test. So he does the test on the whole scaffold dry, and then he does the test on the whole scaffold wet.
And I assume there's some sort of bonding that gets disrupted by having the water. I don't know the details of how that works, but there must be some change in the bonding to make that happen. So let's see. I'm trying to see. What else should we do today?
Oh, yeah. So we get pretty good agreement with this sort of simple foamy model. One of the things we did find was that when we tested higher-density scaffolds, the agreement wasn't so good. And I think this was because when you get higher density, it's just hard to get the collagen GAG mixture to mix in with the acetic acid. And so we ended up getting inhomogeneous scaffolds with sort of large voids in them. So in order for the modeling to work, you have to have a scaffold that's relatively homogeneous. You don't have kind of big defects in it. I think that's all I'm going to say about that.
I have one more slide here. So those are sort of three-dimensional scaffolds we've been talking about. Sort of foam-like scaffolds. People have also made honeycomb-like scaffolds as well. And this just shows some examples of some honeycomb-type scaffolds.
So this one here, I think was made in Sangeeta Bhatia's lab. You can sort of think of it as a hexagon, but it's also kind of triangulated as well.
These two here were made by George Engelmayr. He worked with Bob Langer at one point. And these two scaffolds here, they're both sort of rectangular cells. And they were designed to look at how the cell geometry or the pore geometry affected the sort of morphology of the cells that attached.
So if you have different, say, [? porous, ?] you get different morphology in the cells that you're trying to attach. And then, George Engelmayr also made these scaffolds here. And those were designed to be anisotropic and have different mechanical properties in different directions.
And I think what they had done was try to match the anisotropy in the mechanical properties to anisotropy in heart tissue, in cardiac tissue. So these are some examples of honeycomb-type scaffolds. So let me just write down a few things about those.
So I think these were more-- obviously, the scaffolds are used-- the ultimate goal is to use them clinically. But sometimes, people make scaffolds just to study cell behavior. And some of these, I think, were made just to study how the cells would behave on them. So they're sort of idealized to do that.
So that triangulated. It kind of looks like a hexagon, but there's also sort of triangles in there. I think the thing they were looking at with that was transport of nutrients to cells.
And from a mechanical point of view, if it's triangulated you'd expect, say, the modulus of that to go linearly with how much solid there is there. So the rectangular honeycomb and the diamond shaped pores, they were used to study the effect of pore geometry on the cell orientation. They used fibroblasts. And I'm going to call it the accordion-like honeycomb. That's mechanically anisotropic. And the mechanical anisotropy is matched to the cardiac tissue. OK.
So I think I'm going to stop there for today. That's sort of the end of this part. And next time, I'm going to talk about the osteochondral scaffolds. I don't think it really makes sense to start it for two or three minutes. So I'll start that tomorrow. Or Wednesday. And we should be able to finish that on Wednesday.
So one of the things that these honeycomb-type scaffolds kind of suggests is that the scaffolds are used both to try to regenerate tissue in the body in clinical applications, but they're also used as sort of an environment for cells, in order to study cell behavior. So next time, I'm going to talk about an osteochondral scaffold.
And the idea with that was to try to use it clinically. But next week, I'm going to talk about cell mechanics a bit. And when people study cell mechanics, or look at the mechanics of biological cells, not the cellular structures. So when people look at trying to study how cells behave, they need some environment to put them on. Typically, people started by just using flat 2D substrates. But the flat 2D substrates are kind of easy to study, but they don't really represent the tissue in the body. And so people are now using tissue engineering scaffold as an environment that they can control to study how cells behave. So they study cell attachment, cell proliferation, cell migration, and cell differentiation all by using the scaffolds as kind of a controlled environment. So we'll talk about that, probably not-- I don't know if we'll get to it on Wednesday. But we might start it on Wednesday and finish it next week. OK?
Free Downloads
Video
- iTunes U (MP4 - 162MB)
- Internet Archive (MP4 - 162MB)
Subtitle
- English - US (SRT)